| Front page | | Contents | | Previous | | Next |
Dry deposition and spray drift of pesticides to nearby water bodies
Appendix C. Derivation of the basic equation for the surface resistance of water
References
In this appendix the surface resistance rc for water bodies will be derived. The exchange of gases between the atmosphere and streams, lakes and seas has traditionally been described in a way that is more familiar to engineers than to atmospheric scientists. In stead of describing the exchange with the resistances as atmospheric scientists do they describe the exchange with mass transfer coefficients. For that reason we will describe the engineering approach of exchange here first and then we will see how the derived mass transfer coefficients can be translated into resistances. The physics and chemistry of the processes involved are of course the same for both approaches, only the way they are described is different.
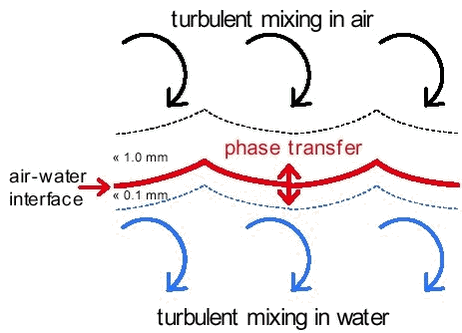
Figure C-1. Model for air-water exchange. On both sides of the air-water interface there is a stagnant or intermittently mixed boundary layer (about 1 mm thick in the air and about 0.1 mm thick in the water). The remaining parts of the air and the water are assumed to be well mixed by turbulence. Phase transfer takes place at the air-water interface. Figur C-1. Model for udveksling mellem atmosfæren og vand. På begge sider af overfladen befinder sig et stagnerende eller periodisk blandet grænselag (ca. 1 mm tyk i atmosfæren og ca. 0.1 mm tyk i vand). Det antages, at resten af atmosfæren og vandet er vel opblandet pga. af turbulens.
Fig.C-1. visualises the processes that play a role in the exchange of gases between the atmosphere and water ( Schwarzenbach, 1993): 1) Turbulent transport in the atmosphere by turbulence to a stagnant or intermittently mixed boundary layer. 2) Transport through a stagnant or intermittently mixed boundary layer of air, which has a thickness of the order of 1 mm. 3) Transport across the air-water interface. 4) Transport through the stagnant or intermittently mixed boundary layer of water, which has a thickness of the order of 0.1 mm. 5) Turbulent transport by turbulence in the water.
The mass transfer from the atmosphere to the water and vice versa depends in principle on the speed for each subsequent step. There are different models available to describe this situation (Schwarzenbach et al., 1993). a) The stagnant two-film model. In this model it is assumed, that the transport through the two stagnant boundary layers occurs by molecular diffusion. As a result, the transport velocity through these layers increases linearly with the diffusivity of the gas in air or water. b) The surface-renewal model. In this model it is assumed that there is a continual turnover of air and water parcels with the (dissolved) gas within the intermittent boundary layers. As a result the transport through the layers increases with the square root of the diffusivity of the gas in air or water (Schwarzenbach et al., 1993).
The main difference between the results of these models is the fact that the transport velocity through the stagnant or intermittent layer increase with the diffusivity in model a) and with the square root of the diffusivity in model b).
It should be noted that many of the parameters that are important in such models like the thickness of the stagnant or intermittent layer cannot be measured, nor can the rate at which the surface is renewed in the surface renewal model. In practice this means that both models include fitting parameters that when appropriately adjusted yield exchange rate estimates that are in agreement with experimental values. It should also be noted that the basic equations for exchange are the same in the stagnant two-film model and the surface-renewal model (Schwartz, 1992; Schwarzenbach et al., 1993). It is only the parameterization of the exchange rate that is different. In the following the basic equations are derived.
In the basic equations concentrations are either expressed in the gas phase or in the aqueous phase. In case of equilibrium gas phase and aqueous phase concentrations are related through Henry’s law:

It should be noted here, that there are numerous definitions of Henry’s law coefficients, having different units and even different senses. In the model it is assumed that that the bulk of the gaseous and aqueous phases is homogeneously mixed. In the following equations are given for the fluxes in each step.
The flux (Fg in kg m-2 s-1) from the bulk gas phase through the stagnant or intermittent layer in the atmosphere to the interface is described by:

where: kg = gaseous phase mass transfer coefficient (m s-1) cg = concentration in the bulk gas phase (kg m-3) cg,i = concentration in the gas phase at the air-water interface (kg m-3)
This equation states that the flux depends on a speed, also called mass transfer coefficient kg and the difference in concentration between the bulk gas phase and the interface. Hence it will depend on the concentration differences in which way (to or from the water) the transport will go.
The flux (Fi in kg m-2 s-1) across the interface is described by:

where: v = mean molecular speed of the gas molecule (m s-1); v cg,i/4 is the gas kinetic collision rate α = mass-accommodation coefficient (dimensionless). This is the probability that a collision at the interface will result in interfacial mass transfer. cw,i = concentration in the aqueous phase at the air-water interface (kg m-3)
This flux depends on the concentration differences between both sides as well as on the molecular speed of the molecule and how big the change is that a collision will result in a transport across the interface. KH is just used to “translate” an aqueous phase concentration in a gas phase concentration, because otherwise concentrations cannot be compared.
The flux (Fw in kg m-2 s-1) from the interface through the stagnant or intermittent layer in the water to the bulk aqueous phase is described by:

where:
ß = factor by which the aqueous phase mass transfer enhances due to removal of the material by chemical reaction. If there is no enhancement ß is 1. If there is enhancement ß will be larger than 1. kw = aqueous phase mass transfer coefficient (m s-1) cw = concentration in the bulk aqueous phase (kg m-3)
This equation indicates that the flux depends on a speed, also called mass transfer coefficient kw and the difference in concentration between the interface and the bulk water phase. Hence it will depend on the concentration differences in which way (to or from the air-water interface) the transport will go.
After a very short time a steady state situation will be reached, where the fluxes Fg, Fi and Fw are equal to each other. The concentrations cg,i and cw,i at the interface are generally not known. For that reason it is useful to express the fluxes in the bulk concentrations cg and cw. This can e.g. be done by finding cw,i that still is a function of cg,i from equations (C-3) and (C-4). Then substituting the expression for cw,,i in equation (C-4) and then finding cg,i from equations (C-4) and (C-2).
In this way the following equation is found for the flux:

where Kg is the overall gas phase mass transfer coefficient (m s-1), which is defined by:

Equation C-5 is in principle the same as equation (5) in the main report where cg,surface = KHcw.
It should be noted that the flux can be expressed as an overall aqueous phase mass transfer coefficient as well:

where Kw is defined by:

Equations (C-7) and (C-8) are aqueous phase equivalents of (C-5) and (C-6). The terms in (C-6) or (C-8) add like electrical resistances in series, illustrating that the mass transfer is governing by three subsequent transfer processes.
If the mass accommodation coefficient is greater than of the order of 1× 10-4, i.e. that the more than 1 out of 10000 gas molecules are absorbed when they hit the water surface the transport through the interface is so fast that it does not limit the overall transfer (Schwartz, 1992). The mass accommodation coefficient has only been determined for a limited number of more common atmospheric gases that have a low molecular weight (Seinfeld and Pandis, 1998) and not at all for pesticides. So it cannot be excluded that for some pesticides the transport through the interface is so slow that it limits the overall mass transfer. In the following, we will assume that the mass transfer coefficient of pesticides is so high that the second term in (C-6) and (C-4) can be neglected. If this is not correct, the mass transfer will occur at a lower rate than predicted with the simplified forms of (C-6) and (C-4).
In the following the effect of chemical reactions is also neglected and it is hence assumed that ß = 1. In reality, however, reactions can be important for some pesticides, especially those that e.g. dissociate very fast in water.
If there is no reaction and no resistance at the interface (i.e. the mass accomodation coefficient is large enough) (C-6) becomes:

There is in that case a critical Henry’s law coefficient KH,crit for which the gas and aqueous phase mass transfer coefficients are equal:

If KH >> KH,crit the overall mass transfer is controlled by transfer in the aqueous phase and for KH << KH,crit the overall mass transfer is controlled by the transfer gas phase.
The approach in this section is the engineering approach. The mass transfer coefficient in the gas phase kg covers in fact the transport from a certain reference height in a part of the turbulent atmosphere, then through the stagnant or intermittently mixed boundary layer to the interface/surface. In the engineering approach the concentration in the bulk air phase is the same everywhere. Atmospheric scientists have a different approach and allow a vertical concentration gradient in the bulk air that is caused by dry deposition.
Meteorologists use the following equation for the flux:

where (see section 2.4 of the main report): ra = aerodynamic resistance (s m-1) rb = laminar boundary layer resistance (s m-1) rc = surface resistance (s m-1) cg,r = concentration in the gas phase at reference height (kg m-3)
Comparing (C-11) for the situation where rc is zero with (C-2) describing the same situation leads to the following equation:

Comparing (C-11) with (C-5) with the Kg value from (C-9) leads to the equation for the surface resistance:

This relation shows that rc increases with KH and decreases with the mass transfer coefficient in the aqueous phase, which is a measure of how fast the compound is transported away from the interface into the bulk water. The molecules that have been transported away from the surface can then again be replaced by “fresh” molecules that reach the interface from the gas phase. It is equation C-13 that we will use in the main report to calculate rc.
It should be noted here that the model not only describes dry deposition to water surfaces, but also emission from water surfaces, e.g. emission that can occur due to spills of chemicals.
References
Schwartz, S.E. (1992). Factors governing dry deposition of gases to surface water. In: Schwartz, S.E. and Slinn, W.G.N. Precipitation scavenging and atmosphere-surface exchange. Hemisphere Publishing Corporation, Washington D.C., USA, 789-801.
Schwarzenbach, R.P., Gschwend, P.M., Imboden, D.M. (1993). Environmental organic chemistry. John Wiley, New York, USA.
Seinfeld, J.H., Pandis, S.N. (1998) Atmospheric chemistry and physics. John Wiley, New York, USA.
| Front page | | Contents | | Previous | | Next | | Top |
|