| Front page | | Contents | | Previous | | Next |
Appendices 1-18 to: Report on the Health Effects of Selected Pesticide Coformulants
65 Toxicokinetics
65.1 Absorption, distribution, and excretion
65.1.1 Inhalation
65.1.2 Oral intake
65.1.3 Dermal contact
65.2 Metabolism
65.3 Mode of action
65.1 Absorption, distribution, and excretion
65.1.1 Inhalation
In nose-only exposure experiments, rats were exposed to 14C-ethylene glycol vapour (32 mg/m3) for 30 minutes or to an ethylene glycol aerosol (184 mg/m3) for 17 minutes. Estimates indicate that at least 60% of inhaled ethylene glycol from both exposures was deposited in the nasal cavity. Absorption and distribution from the site of deposition was rapid, since in animals, which were sacrificed immediately after exposure, 75-80% of the received dose had already been distributed in the animal’s body. The half-time for clearance of plasma 14C-activity (ethylene glycol plus metabolites) was 39 hours for ethylene glycol vapour and 34 hours for the aerosol (Marshall & Cheng 1983 – quoted from BUA 1991, NTP 1993).
65.1.2 Oral intake
The approximate serum half-life of ethylene glycol has been reported to be 2.5 hours in children (Rothman et al. 1986 – quoted from ATSDR 1997) and between 3.0 and 8.4 hours in adults (Jacobsen et al. 1988, Peterson et al. 1981 – both quoted from ATSDR 1997).
Reif (1950 – quoted from Ware 1988), on three separate occasions, drank pure ethylene glycol in 100 ml of water. The amounts consumed were 5.5, 11.0 and 13.2 g (corresponding to 78.5, 157, and 188.6 mg/kg, respectively, assuming a body weight of 70 kg). Ethylene glycol was recovered in the urine at 24 to 31% of the administered dose within 24 to 48 hours. Oxalic acid concentrations in the urine were higher than normal for 8 to 12 days, with a peak on the fourth day.
In rats, ingested ethylene glycol is rapidly absorbed and evenly distributed throughout the body reaching peak blood levels at 1 to 4 hours after ingestion of doses of 7-29 mg/kg (Winek et al. 1978 – quoted from ATSDR 1997).
The kinetics of orally administered ethylene glycol and its major metabolites, glycolic acid and oxalic acid, in pregnant rats were compared across doses, and between pregnant (P) and non-pregnant (NP) rats. Groups of rats were administered 13C-ethylene glycol by gavage at doses of 10 (P and NP), 150 (P), 500 (P), 1000 (P), or 2500 (P and NP) mg/kg b.w. (Pottenger et al. 2001).
Pregnancy status (gestation days 10-11) had no significant impact on the blood concentration-time profiles of ethylene glycol, glycolic acid, or oxalic acid. Thus, the kinetic parameters estimated (maximal concentration in blood (Cmax), time to reach maximum blood levels (Tmax), area-under-the curve (AUC), and half-life of elimination (bt½)) did not differ significantly between the pregnant and non-pregnant groups.
The Tmax for ethylene glycol for all dose groups occurred at one hour after dosing; the blood concentration decreased in a log-linear fashion thereafter and was no longer detectable for the low dose group (10 mg/kg) by 12 hours post-dosing and by 24 hours for the 150 and 500 mg/kg dose groups. The estimated half-time for elimination of ethylene glycol from blood was less than 2 hours for all dose levels. Blood levels of glycolic acid increased to a peak at 3 hours post-dosing, except at the lowest dose level (10 mg/kg) where this metabolite could not be detected in the blood. The blood levels decreased by 24 hours post-dosing to undetectable levels. The concentrations of oxalic acid in the blood varied between undetectable and about 2 times the limit of quantification over the 24-hour collection period.
There were no substantial differences in the urinary elimination profiles between the pregnant and non-pregnant dose groups, at comparable dose levels. Urinary elimination of ethylene glycol and its metabolites demonstrated dose-dependency, with the high dose groups (2500 mg/kg) eliminating almost 70% of the administered dose in urine, compared with about 16% in the low dose groups (10 mg/kg). The shift in urinary elimination was mainly due to increased urinary glycolic acid and ethylene glycol, and not to increased elimination of oxalic acid.
In rats, 10-20% of oral doses up to 1000 mg/kg of ethylene glycol were recovered from the body tissues and carcass 96 hours after dosing, whereas mice retained only a small percentage of the dose in their tissues. Total recovery of the oral doses in rats and mice was approximately 90-100%, indicating substantial absorption. The major excretory route of [14C] was via exhalation of carbon dioxide (42%), while 24% of the dose was excreted via the urine and 3% via the faeces. (Frantz et al. 1989, 1991 – quoted from ATSDR 1997).
The elimination half-life in plasma has been estimated at 1.7 hours in rats given 2000 mg/kg and 1.4 to 2.5 hours following administration of 10 to 1000 mg/kg (Frantz et al. 1989 – quoted from ATSDR 1997).
Mice showed a similar profile, exhaling 55% of the dose, and excreting 24% in the urine and up to 12% in the faeces. The elimination half-life in plasma in mice has been estimated at 0.3 to 1.1 hours following administration of 10 to 1000 mg/kg. (Frantz et al. 1991 – quoted from ATSDR 1997).
In contrast, approximately 50% of an oral dose of ethylene glycol administered to dogs was excreted via the urine (Grauer et al. 1987 – quoted from ATSDR 1997).
65.1.3 Dermal contact
The in vitro permeability of human skin to ethylene glycol was determined by Loden (1986 – quoted from ATSDR 1997); the rate of resorption was 118 µg/cm2/hour, with a steady state concentration of 0.97 mg/cm2.
14C-ethylene glycol (in acetone vehicle) was applied to the surface of three different fresh human skin samples at a dose of 8 µg/cm2. After 24 hours of exposure, 18.3% of the applied dose was recovered in the receptor fluid (absorbed through the skin), 8.3% in the skin, and 12.5% in the skin surface wash (total accountability was approximately 39%). Individual differences existed for the three samples; average absorption was 26.6% relative to the 8 µg/cm2 applied dose for a 24-hour exposure duration. This represented a flux of approximately 0.09 µg/cm2/hour for ethylene glycol. The maximum flux observed was 0.25 µg/cm2/hour. (Driver et al. 1993).
In dermal applications using an occlusion bandage, approximately 30% of doses of ethylene glycol up to 1000 mg/kg was absorbed through rat skin; 14% of the absorbed dose was expired, while 7% was excreted in the urine, and 1% was recovered from the faeces (Frantz et al. 1989 – quoted from ATSDR 1997).
Following administration of 100 mg/kg undiluted radiolabeled ethylene glycol to mice using an occlusive bandage, 99.5% of the dose was recovered, with tissues and excreta accounting for 76.5%. Most was recovered as volatile organic radioactivity (25-39%) or as radioactive carbon dioxide (8-12%). Urine and faeces each accounted for another 4.9% of the dose. Tissue recoveries were less than 1% of the dose, while the residual carcass contained about 10-18% of the dose. Following application of 1000 mg/kg undiluted ethylene glycol or as an 50% aqueous solution, the total recovery was 89% of the dose with 84% in tissues and excreta, and approximately 7% in faeces, cage wash water, and carcass. According to the authors, mice absorbed 85-100% of the administered dermal dose. (Frantz et al. 1991 – quoted from ATSDR 1997).
65.2 Metabolism
The metabolic pathway for ethylene glycol is shown in Figure 2.2. Solid arrows represent the steps that are quantitatively most important, while the broken arrows indicate minor metabolic conversions in humans. (ATSDR 1997).
Ethylene glycol is oxidised to glycolaldehyde by NAD-dependent alcohol dehydrogenase in the liver and kidney. Glycolaldehyde is further oxidised to glycolic acid by mitochondrial aldehyde dehydrogenase and cytosolic aldehyde oxidase. There is no evidence for an accumulation of glycolaldehyde and it appears to be rapidly metabolised to glycolic acid (Jacobsen & McMartin 1997).
A small amount of glycolaldehyde is converted to glyoxal which, in presence of lactate dehydrogenase and/or aldehyde oxidase, is further converted to glycolic acid or is directly metabolised via an oxidative mechanism to glyoxylic acid.
Further degradation of glycolic acid is oxidation to glyoxylic acid by glycolic acid oxidase or lactic dehydrogenase. This step occurs apparently at a slow rate since glycolate has been shown to accumulate in large amounts (Jacobsen & McMartin 1997).
Glyoxylic acid is further metabolised via a number of intermediate metabolic pathways, for example, to oxalic acid by the enzyme glycolic acid oxidase and, via formic acid, to carbon dioxide and water, or to glycine. The major metabolic route in terms of toxicological importance is the conversion to oxalate and the most important alternate pathway appears to be formation of glycine (Jacobsen & McMartin 1997). Glyoxylate can induce lactic acid formation via oxalomalate production and its inhibitory effects on the citric acid cycle.
(ATSDR 1997, Jacobsen & McMartin 1997, BUA 1991, Cavender & Sowinski 1994).
65.3 Mode of action
The toxicity of ethylene glycol is mainly a result of the effects of its metabolites
such as glycolaldehyde, glycolic acid, glyoxylic acid, and oxalic acid. (ATSDR 1997, Jacobsen & McMartin 1997, LaKind et al. 1999, BUA 1991, NTP 1993).
The clinical symptoms of acute ethylene glycol poisoning in humans can be divided into three (and occasionally four) fairly distinct stages (see also part 3.1.2) (LaKind et al. 1999, Cavender & Sowinski 1994, BUA 1991, ACGIH 2001).
The first stage, which usually begins within 30 minutes to 12 hours after ingestion, consists primarily of central nervous system (CNS) toxicity and is usually attributed both to unmetabolised ethylene glycol and to the formation of aldehydes that peak 6 to 12 hours after ingestion (LaKind et al. 1999). The appearance of cerebral symptoms coincides with the highest concentration of the metabolite, glycolaldehyde (Balazs et al. 1982 – quoted from BUA 1991).
The second stage has been described as the cardio-pulmonary toxicity stage that occurs 12 to 72 hours after ingestion. This stage may also be characterised by severe metabolic acidosis. (LaKind et al. 1999).
Aldehyde intermediates are held responsible for the cytotoxic effects and glycolaldehyde seems to be responsible for the observed cardio-pulmonary symptoms (Balazs et al. 1982 – quoted from BUA 1991).
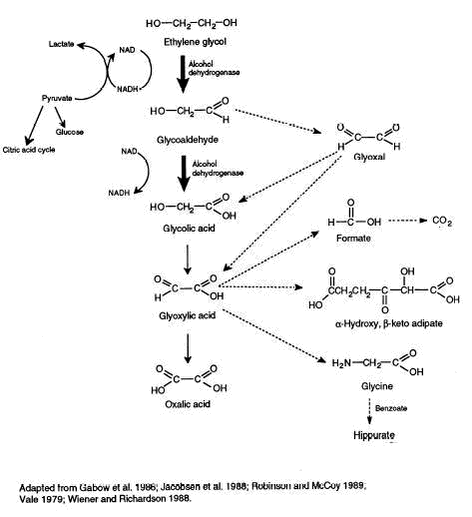
Figure 2.2. Metabolic pathway of oxidation of ethylene glycol. From ATSDR (1997).
Remark: Glycolaldehyde is oxidised to glycolic acid by aldehyde dehydrogenase, not alcohol dehydrogenase as stated in the figure.
The third stage is known as the “renal failure” stage and occurs 24 to 72 hours after ingestion. This stage ischaracterised by profound acidosis caused, according to LaKind et al. (1999), primarily by the accumulation of the metabolites glycolic acid and glyoxylic acid.
Recent studies of cases of human ethylene glycol poisoning have demonstrated that the major determinant of the metabolic acidosis is the degree of glycolic acid accumulation and glycolate accumulation correlates with the increase in anion gap or decrease in arterial bicarbonate concentrations in humans, as well as in animals. Several studies have suggested accumulation of glyoxylate, although at much lower levels (60 µM) than that of glycolate (15 mM). Glycolate is less toxic in vitro than either glycolaldehyde or glyoxylate. Whether the levels of glyoxylate that have been measured in human cases can contribute to the clinical features cannot yet be determined. (Jacobsen & McMartin 1997).
Calcium oxalate precipitation within the renal tubules has long been accepted as an important pathogenic factor for the development of renal toxicity; however, the mechanism of the renal toxicity is not yet known and there is no evidence directly linking oxalate precipitation with development of renal tubular necrosis. Furthermore, the renal damage can occur at levels of exposure where no or few crystals of oxalate are detected. Therefore, the renal toxicity has also been suggested to occur from a metabolite-induced cytotoxicity. (Jacobsen & McMartin 1997, LaKind et al. 1999).
Glycolic acid and glyoxylic acid react with bicarbonate causing a decrease in the pH in body fluids, particularly in blood. An elevated anion gap develops, and the serum osmolal gap across cells increases, resulting in renal oedema that compromises intrarenal blood flow and promotes renal failure. Blood phosphorous (inorganic) levels are increased due to uncoupling of oxidative phosphorylation, and, as a result, blood calcium levels are lowered. Acidosis results in oliguric or anuric renal failure with renal changes. (LaKind et al. 1999).
Generally, the role of oxalate accumulation in the toxicity of ethylene glycol has not been clarified. Urinary calcium oxalate crystals are an important hallmark of ethylene glycol poisoning. However, plasma oxalate determinations in human cases have showed low levels (< 0.4 mM) possibly because the oxalate in plasma rapidly precipitates as calcium oxalate, whose crystals have been noted in the kidney, brain and other tissues. Such precipitation probably leads to the hypocalcaemia that is characteristic of ethylene glycol poisonings. (Jacobsen & McMartin 1997).
Other neurological symptoms, effects on cranial nerves, have been identified 6 or more days after ingestion and constitute a possible fourth clinical stage of toxicity. The mechanism(s) of these delayed neurological effects is unknown, but is distinctly different from the pathological events of the first three stages. The cause may be related to oxalate crystal deposition or, perhaps, ethylene glycol may induce pyridoxine deficiency, resulting in peripheral neuropathy. (LaKind et al. 1999).
The mechanism(s) of ethylene glycol toxicity as related to developmental effects warrants further exploration. A link between maternal metabolic acidosis and developmental toxicity has been suggested. (LaKind et al. 1999).
Pottenger et al. (2001) has suggested that glycolic acid is the proximate developmental toxicant.
| Front page | | Contents | | Previous | | Next | | Top |
|