| Front page | | Contents | | Previous | | Next |
Background for spatial differentiation in LCA impact assessment - The EDIP2003 methodology
6 Photochemical ozone formation
6.1 Introduction
6.1.1 Precursors and photo-oxidants
6.1.2 Photochemical ozone formation scheme
6.2 Existing systems for characterisation of ozone formation
6.3 The Rains model
6.3.1 Modelling ozone formation
6.3.2 Exposure indices in RAINS
6.3.3 Validation of the RAINS model
6.4 Spatially differentiated characterisation factors
6.4.1 Sub categories of ozone formation
6.4.2 Characteristics of the new characterisation factors
6.4.3 Differentiation between individual VOCs
6.5 How to perform site-generic and site-dependent characterisation
6.6 Normalisation references
6.7 Interpretation and recommendations
6.8 References
Annex 6.1 Influence from meteorological conditions
Annex 6.2 POCP values for individual VOCs
Annex 6.3 Efficiency factors for individual VOCs and source-specified VOCs
Annex 6.4 Normalisation references for photochemical ozone formation
Authors: Michael Hauschild [44]Annemarie Bastrup-Birk [45] Ole Hertel45 Wolfgang Schöpp [46] José Potting44
6.1 Introduction
The concentration of ozone in the troposphere rises by about 1% a year over the Northern hemisphere. An important source of this ozone as well as of other reactive substances is the photochemical
oxidation of volatile organic compounds and carbon monoxide in the presence of nitrogen oxides and sun light in the boundary layer of the troposphere. The troposphere is the lower stratum of the
atmosphere, reaching from the surface of the earth to the tropopause 8-12 km above us and the boundary layer is the lower part of the troposphere (up to an altitude of around 1 km) where full mixing can
be assumed and where the exposure of life to the photo oxidants takes place. Due to their reactive nature, the photo-oxidants are injurious to the health of living organisms, vegetation as well as animals and
humans. Apart from the general increase in the tropospheric ozone concentration, smog-episodes can occur on a more local scale in cities given a combination of high emission levels and the right
meteorological conditions. During smog-episodes, the concentrations of ozone and other photo-oxidants reach extreme levels causing immediate damage to human health.
Due to the local and discontinuous nature of photochemical smog-episodes, the focus in life cycle impact assessment is mainly on the more regional increase of tropospheric ozone.
6.1.1 Precursors and photo-oxidants
The precursors necessary for photochemical ozone formation are nitrogen oxides which have a kind of catalytic effect and volatile organic compounds which are oxidised during the process.
While NOx, nitrogen oxides, represents the sum of nitric oxide NO and nitrogen dioxide NO2, there is no universal definition of what a volatile organic compound, VOC is. In the current context, it is
defined as an organic compound with a boiling point below 250C (WHO, 1989). For a VOC to contribute to photochemical ozone formation it must either contain hydrogen (i.e. it can not be fully
substituted), or contain double bonds (i.e. be unsaturated) (Hauschild and Wenzel, 1998). Methane, which is a VOC according to the definition, is generally treated separately from the rest of the VOCs due
on the one side to its very low photochemical reactivity (as reflected in its extremely long atmospheric residence time), and on the other side its large proportion of the total emission of volatile organic
compounds. The abbreviation VOC is therefore generally – and also throughout this chapter - used to characterise the volatile organic compounds apart from methane (in some contexts also designated the
NMVOCs).
While being an intermediate in the atmospheric oxidation of VOCs, carbon monoxide from man-made emissions also contributes to photochemical ozone formation.
The photo-oxidants comprise a variety of unstable, oxidising substances formed when VOCs react with oxygen compounds which are naturally present in the troposphere (the most important being the
hydroxyl radical, OH). Among the photo-oxidants, the most prominent are ozone, O3, and peroxyacetyl nitrate, PAN, but intermediate products from the oxidation of VOCs also contribute to the injurious
effects. It is thus not the VOCs per se which cause the environmental problems associated with photochemical ozone formation, but the products of their conversion. If a direct toxic effect of VOCs occurs,
it is treated separately in life cycle impact assessment as a contribution to the impact categories human toxicity and ecotoxicity.
6.1.2 Photochemical ozone formation scheme
Some tropospheric ozone arises from the natural transport of ozone from the stratosphere, where it is formed by the action of energetic UV rays from the sun, but the rest is produced in the troposphere by
the photochemical ozone formation. Schematically, the photochemical ozone formation can proceeds through the following four steps:
1. Reaction between VOCs or CO and OH to form peroxy radicals, ROO.
2. The peroxy radicals oxidise NO to NO2.
3. NO2 is split by sunlight with formation of NO and release of oxygen atoms.
4. Oxygen atoms react with molecular oxygen, O2, to form ozone.
The reaction schemes are complex and difficult to simulate in a model. The heterogeneous spatial distribution of VOC and NOx sources across Europe, and the many hundreds of chemical species involved,
makes the photochemical formation of ozone on a regional scale highly non-linear.
Apart from the emitted quantity of VOC and NOx, the following factors influence the ozone formation:
Meteorological conditions
Solar radiation is a driving force behind the photo oxidation but also wind speeds and directions (determining the dispersion of the air pollutants), temperature (influencing reaction kinetics) and precipitation
patterns (influencing wet deposition and hence removal of pollutants from the boundary layer) are conditions that influence the photochemical ozone formation
Interaction between pollutants
The simultaneous concentration of the other relevant pollutants, NOx and individual VOCs, can strongly influence the overall result. The dependence of ozone formation on the precursor emissions (NOx,
VOC) is therefore often far from linear.
In regions with relatively low emissions, the NOx/VOC ratio is generally low, and ozone formation is limited by NOx. In these regions, reduced NOx emissions will thus reduce ozone formation while
reductions in VOC emissions may have little influence on the ozone formation.
In regions with extremely high NOx emissions, reduction in the emissions may actually lead to increased ozone formation. Here, the high concentrations of NO may lead to reduction of ozone (through
oxidation of NO to NO2). In addition, NO may reduce the concentration of hydroxyl radical which thus limits the potential for oxidation of VOCs. In this situation, reduced emissions of NOx will therefore
increase the availability of hydroxyl radical, increasing the oxidation of VOC and hence the ozone formation, unless VOC emissions are reduced at the same time. (Heyes et al., 1997a).
Biogenic emissions of VOCs
In Europe, the total natural emissions of biogenic VOCs is thought to be around 30 % of the total VOC emissions (Derwent et al., 1991) and this must be represented in a model for ozone formation. For the
biogenic (natural) releases of NMVOC only little information is presently available, and often the empirical expressions derived by Lübkert and Schöpp (1989) to describe releases from forested areas are
therefore applied.
Properties of the individual VOC
As mentioned earlier, the photochemical reactivity of methane is much lower than the reactivity of the VOCs. Among the VOCs there is also variation in the potential for formation of ozone as reflected in the
individual photochemical ozone creation potentials (POCP) shown in Annex 6.2.
A ranking of the different groups of VOCs according to their ozone formation potential per unit weight results in the following sequence (Derwent and Jenkin, 1990):
- Alkenes (reactivity decreasing with chain length) and aromatics (reactivity increasing with the degree of alkyl substitution, decreasing with the length of the chain in the substituted alkyl group).
- Aldehydes (the strongest is formaldehyde; benzaldehyde has no or even a negative ozone formation potential).
- Ketones
- Alkanes (reactivity almost constant from C3 upwards), alcohols and esters (the more substituted the lower reactivity).
- Halocarbons (reactivity decreasing with the degree of halogen substitution).
6.2 Existing systems for characterisation of ozone formation
Figure 6.1 shows the cause-impact chain for photochemical ozone formation. The descriptors provided for each link of the chain are characteristics or parameters which influence the behaviour at that point
in the chain.
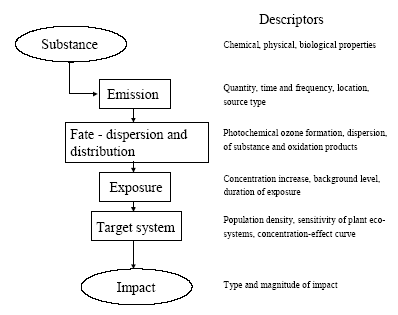
Figure 6.1 Cause-impact chain for photochemical ozone formation.
The existing characterisation factors for photochemical ozone formation are all based on the photochemical ozone creation potential, POCP or possibly the Maximum Incremental Reactivity (MIR) of the
substances. Both express the short-term contribution to ozone formation. MIR is primarily designed to reflect the contribution during photo-smog episodes while POCP may be calculated with time horizons
from 1 to 9 days. While MIR is expressed directly as moles of ozone produced per mole of VOC emitted, POCP is expressed as an equivalent emission of the reference substance ethylene (C2H4) – a
strong ozone formation gas.
In EDIP97, photochemical ozone formation is characterised using POCP-values for ozone formation over 4-9 days. Some spatial differentiation is built into the EDIP97 through a distinction according to the
NOx concentration in the emission area. There are thus two sets of characterisation factors – one for low NOx regions and one for high NOx regions. This is a rather rough distinction since emissions taking
place in high NOx regions may well be transported to low NOx regions and oxidised there (and vice versa) but at that time it was considered by the authors to be the best representation of the spatial
variability of photochemical ozone formation that would be feasible in life cycle impact assessment.
In addition to POCP values for around 90 individual VOCs, EDIP97 also provides aggregated POCP values for some common VOC-mixtures identified by their source rather than their composition.
Furthermore, tools are provided for estimation of missing POCP values.
Both the POCP and the MIR address impacts at the level called fate-dispersion and distribution in Figure 6.1. They reflect the substance's intrinsic potential for ozone formation under a set of standard
conditions regarding the presence of other gases and the meteorological conditions and they focus primarily on the ozone formation during the first days after the emission as relevant for the prediction of
photo smog episodes.
The behaviour through the first links of the cause-impact chain from emission to the point where the POCP and MIR are modelled, depends to a large extent on the properties of the substance. Further along
the chain, the conditions of the environment where the substance is emitted, dispersed, oxidised and where exposure of sensitive target organisms takes place become decisive. Hitherto, it has been seen as
impossible to include these descriptors in the modelling of impact, even though they are known to be important – for photochemical ozone formation perhaps more important than the descriptors in the early
parts of the cause-impact chain as illustrated by the spatially differentiated factors developed in Section 6.4. It has not been feasible to perform the required spatial differentiation in life cycle assessment, and
therefore a low environmental relevance of the modelled impacts has been accepted.
For photochemical ozone formation, an additional problem about the existing characterisation models is their inability to represent the impact from NOx. Both POCP and MIR have been developed for
ranking of VOCs according to their ozone formation potential and the presence of NOx is considered a prerequisite. Due to the lack of factors for NOx, its contribution to ozone formation is neglected in
current life cycle impact assessment and this is a serious flaw as seen from the normalisation references calculated in Section 6.6 showing that the contribution from NOx could be around 2/3 of the total
ozone formation in Europe.
It has been the purpose of the work performed under the Danish LCA methodology and consensus-creation project to include as much as possible of the later links of the cause-impact chain including this
spatially determined variation. The aim has been to include exposure assessment in the characterisation without losing the feasibility in LCIA.
In the endeavour to meet these objectives, an integrated impact assessment model was found which supports quantification of the ozone formation potential of both VOC and NOx in a site-dependent
manner allowing the development of characterisation factors which include the later links in the cause-impact chain - both exposure assessment and parts of the target system description. This model is
described in Section 6.3
6.3 The Rains model
The RAINS (Regional Air Pollution Information and Simulation) model is an integrated assessment model that combines information on regional emission levels with information on long-range atmospheric
transport in order to estimate patterns of deposition and concentration for comparison with critical loads and thresholds for acidification, eutrophication and tropospheric ozone formation. One of the main
objectives with the RAINS model is to establish the relationship between the site of an emission and the impact on its deposition areas. The RAINS model (version 6.2) has therefore been seen as an
obvious choice to establish spatially differentiated characterisation factors for photochemical ozone formation in parallel to what has been done for acidification (Chapter 3) and terrestrial eutrophication
(Chapter 4). Alcamo et al. (1990) and Amann et al. (1995) provide a detailed description of the general model and Section 3.3 gives a summary of those parts of RAINS which are relevant for its use in our
context.
6.3.1 Modelling ozone formation
RAINS is intended to support the development of cost-effective European abatement strategies for different types of air pollution. For photochemical ozone, the focus is on the long-term ozone levels at the
more regional scale. For this purpose, it is crucial with an integrated assessment that represents the relationship between source and receptor regions. For the modelling of ozone formation, RAINS does not
apply a mechanistic model of the highly complex reaction schemes behind the formation of ozone and other photo-oxidants. Such models are used for calculation of POCPs but they will not be operational in
an integrated assessment model. Instead, RAINS builds on a computationally efficient `reduced-form' model of ozone formation using statistical methods to summarise the response to emission changes given
by a more complex reference model, (Heyes et al., 1997b).
In the `reduced-form' model as presented in Heyes et al. (1997a), the long-term mean ozone concentration in any one grid cell j is expressed as a function of the annual non methane VOC emission vi and
the annual NOx emission ni from emitter country i and the effective NOx emission enj experienced in grid cell j over the period considered (enj is corrected for exchange with the free troposphere):
(6.1)
Click here to see the Figure.
where
M is the number of emitter countries considered
kj includes the effects of background concentrations of O3 and its precursors and natural VOC emissions
aijvi provides the linear country-to-grid contribution from VOC emissions in country i (allowing for meteorological effects)
bijni provides the linear country-to-grid contribution from NOx emissions in country i (allowing for meteorological effects)
cijni2 serves as a correction term to allow for non-linearities occurring close to countries with very high NOx emissions
αjenj2 represents the average non-linearity in the O3/NOx relationship experienced along the trajectories arriving at grid cell j and any non-linear effects local to grid cell j
dijenjvi allows for interactions between NOx and VOCs along the trajectories
The coefficients aij, bij, cij and αj are estimated by linear regression using ni, vi and enj as variables in the more complex reference model.
Emission estimates
The resulting factors depend on the emission patterns of European countries. They may therefore vary in time, and in order to reveal temporal variation, the factors are calculated for 1990, 1995 and 2010.
The underlying per country emissions of non methane VOCs and NOx are based on the inventories of the UN ECE for 1990 and 1995 while the 2010 emission scenario is estimated based on the current
reduction plans as expressed by the individual European countries.
Meteorological conditions
To reduce the influence of annual variations in meteorological conditions, the characterisation factors for each of the emission years 1990, 1995 and 2010 are derived as the average of five different
calculations using the meteorological data for the years 1989, 1990, 1992, 1993 and 1994 respectively. Annex 6.1 illustrates the variation between the meteorological years for the calculation of AOT60 for
the emission year 1995
6.3.2 Exposure indices in RAINS
The main target organisms of photo-oxidants are human beings and plants. In RAINS, exposure indices have been developed to quantify the exposure of vegetation as well as humans.
Vegetation exposure
Research on ozone-related vegetation damage makes it possible to define biologically meaningful, but simple, indices to characterise critical ozone exposure and to identify the critical levels of exposure
above which by definition, adverse direct effects on receptors, such as certain plant species, may occur. Critical levels to protect vegetation are best established using long term exposure measures. Based on
the scientific work on critical levels carried out under the UN/ECE Convention on Long-range Transboundary Air Pollution Working Group on Effects, a number of guideline values have been recommended
by WHO (1997). For vegetation, the recommended threshold is 40 ppb and the cumulative exposure index AOT40 – exposure above the threshold of 40 ppb has been accepted as the best available
exposure index for damage to crops and natural vegetation (Kärenlampi and Skarby, 1996).
The concept of accumulated excess ozone assumes a threshold below which no or small effects occur. The AOT40 uses hourly concentrations during daylight hours over a three-month period (growing
season) for herbal plants and crops and a six-month growing period for trees. The AOT40 is calculated as the sum of the differences between the hourly ozone concentrations in ppb and 40 ppb for each
hour when the concentration exceeds 40 ppb, using daylight hours only. It is measured as ppbhours or more commonly as ppmhours.
Under the UN/ECE Convention on Long-range Transboundary Air Pollution, the critical level for exposure of herbal plants and agricultural crops (relating to a five percent crop loss) has been set at an
AOT40 of 3 ppmhours for the growing season and daylight hours, averaged over a 5-year period. For forest trees, a critical level has been set at 10 ppmhours for daylight hours, accumulated over a six
month growing season and also averaged over five years (Heyes et al., 1997b). For the currently prevailing European ozone levels, the critical level for crops and natural vegetation is thus stricter than the
critical level for forest trees. Therefore the critical level for vegetation exposure applied in RAINS, and hence also in the calculated characterisation factors for vegetation exposure, is the level for crops and
natural vegetation.
To reduce the influence from fluctuations in meteorological conditions, the ozone exposure in each grid used for calculation of the indices is determined as an arithmetic average of exposures calculated using
the meteorology for five separate years in the period 1989-1994.
The AOT40 is expressed in two different vegetation-related exposure indices:
a cumulative vegetation exposure index calculated as the excess AOT40 (i.e. the AOT40 in excess of the critical level of 3 ppmhours) multiplied by the area of ecosystems exposed to the excess
concentration
an average vegetation exposure index reflecting the average excess AOT40 in a country.
Both indices are based on rural ozone concentrations (Heyes et al., 1997b)
Human exposure
Ozone reacts with macro-molecules in the tissue of the human air ways. Depending on respiration frequency and ambient ozone concentrations, ozone will react in different parts of the air ways. At high
respiration frequency and low ambient concentrations, ozone will mainly react in the upper air ways, whereas high speed of respired air and high ozone concentrations lead to transport of ozone deep into the
lungs. A detailed Danish review and evaluation of health effects related to traffic air pollution is found in Larsen et al. (1997).
For exposure of human beings, no thresholds for chronic exposure have been established. Following the revised WHO Air Quality Guidelines for Europe (WHO, 1997), a maximum eight-hour average
concentration of 60 ppb is proposed as the long-term environmental objective for the EU ozone strategy. The maximum is calculated from running eight-hour averages of the one-hour mean concentrations.
The ultimate goal would be to eliminate all excess of this criterion. The modelling of European abatement strategies for individual days over a multi-months period is a rather ambitious task and is not entirely
feasible at the moment. In order to simplify the modelling task, the target of no-exceedance of the WHO criterion (60 ppb as maximum eight hours mean concentrations) has been converted into an AOT
index for human health in parallel to the AOT40 index for vegetation damages for use in RAINS. The AOT60 is calculated in the same way but applying the threshold of 60 ppb. As a result, an AOT60 (i.e.,
the cumulative excess exposure over 60 ppb, for practical reasons over a six-month period) of zero is considered as equivalent to the full achievement of the WHO criterion. Any violation of this WHO
guideline will consequently result in an AOT60 of larger than zero.
It is emphasised by the group behind the RAINS model that the interpretation of the AOT60 index should be cautious. Given the current knowledge on health effects it is not possible to link any AOT60
value larger than zero with a certain risk to human health (Heyes et al., 1997b).
Like the AOT40, the AOT60 is expressed in two different exposure indices:
- a cumulative index which reflects the total exposure of a population and is expressed in personppmhours. It is calculated as the product of the average exposure per person and the total population within
the exposed area. The RAINS model calculates these indices on a grid basis (using gridded data on AOT60 and population). Next, these grid values are aggregated to the country level. The cumulative
index in RAINS uses the AOT60 concentrations per grid, representing the rural ozone concentrations, and the total population per grid in 1990.
- an average indicator reflecting the average exposure of a person in a country calculated from the gridded data
Inaccuracies may occur for grids with major urban areas, where the rural ozone concentrations used for these analyses are lower than the concentrations occurring in the city plumes. The `average' indicator
reflects the average exposure of a person in a country, calculated from gridded data.
It is important to stress that these indices may not be used to derive estimates of health damage, for which more detailed information is deemed necessary. In the context of this report, these indices provide
relative measures to enable a comparison of different scenarios.
As for AOT40, ozone exposure in each grid cell used for calculation of the indices is an arithmetic average of exposures calculated using the meteorology for five separate years during the period
1989-1994.
The hourly mean tropospheric concentrations of ozone in Europe vary between 0 and 150 ppb, but generally lie in the range 10 to 40 ppb. The background concentrations in Denmark are around 35 ppb
(Granby et al., 1998). In the northern part of Europe the concentrations are highest in the summer months (May-August). During this period the ozone concentrations thus frequently exceed thresholds for
damages on vegetation and for human health in large parts of Europe.
6.3.3 Validation of the RAINS model
The performance of the reduced-form model for ozone formation has been validated in terms of its calculation of AOT40 against the results of the full EMEP model in Heyes et al., 1996. Over a realistic
range of emissions, it was found to give a very good performance with results on mean ozone concentrations typically deviating less than 1.3% from the full model. A bias appears when the AOT40
approaches zero. In our use, only the excess of 3 ppmhours are counted, so this seems to be a minor problem.
The full model takes into account differences in the composition of the VOC emissions for different sectors. Such differences are not included in the reduced-form model and this is a source of deviation from
the results of the full EMEP model
Overall the performance of the reduced-form model seems satisfactory for our purpose. The reader is referred to Heyes et al., 1996 for further details on the validation.
6.4 Spatially differentiated characterisation factors
The use of RAINS for calculation of spatially differentiated characterisation factors is described in detail in Chapter 3 for acidification. The procedure is similar for photochemical ozone formation with one
main difference. For acidification, the factor for a country is determined by the change in impact caused by a marginal increment of that country's emissions. For photochemical ozone formation, the national
factors have been determined through an analytical solution of the differential quotient of the functional expression for AOT40 or AOT60 respectively.
The functional form of the AOT40-model is the same as the form of the mean ozone concentration model in equation (6.1):
(6.2)

The variables and parameters are explained under equation (6.1).
Site-dependent characterisation factors
The resulting site-dependent characterisation factors are shown in Table 6.1 for exposure of vegetation and in Table 6.2 for exposure of humans.
Table 6.1. Site-dependent characterisation factors for exposure of vegetation to photochemical ozone expressed as cumulative AOT40 in exceedance of the critical level (m2ppmhours/g). Factors calculated
for the emission patterns of 1990 and 1995 and the expected pattern of 2010.
|
NOx |
VOC |
Country/region |
1990 |
1995 |
2010 |
1990 |
1995 |
2010 |
Albania |
1,1 |
1,1 |
1,1 |
0,19 |
0,19 |
0,19 |
Austria |
3,0 |
3,0 |
3,0 |
0,49 |
0,49 |
0,45 |
Belarus |
1,6 |
1,6 |
1,6 |
0,40 |
0,40 |
0,35 |
Belgium |
1,6 |
1,6 |
1,5 |
1,1 |
1,1 |
1,1 |
Bosnia/Herzegovina |
1,6 |
1,6 |
1,5 |
0,22 |
0,22 |
0,20 |
Bulgaria |
1,4 |
1,4 |
1,4 |
0,29 |
0,29 |
0,28 |
Croatia |
2,4 |
2,4 |
2,3 |
0,31 |
0,31 |
0,29 |
Czech Republic |
2,4 |
2,4 |
2,3 |
0,91 |
0,91 |
0,86 |
Denmark |
1,5 |
1,5 |
1,4 |
0,76 |
0,76 |
0,66 |
Estonia |
0,17 |
0,16 |
0,13 |
0,62 |
0,62 |
0,50 |
Finland |
0,41 |
0,38 |
0,33 |
0,29 |
0,29 |
0,25 |
France |
3,4 |
3,4 |
3,3 |
0,88 |
0,87 |
0,75 |
Germany-new |
3,0 |
2,9 |
2,9 |
1,5 |
1,5 |
1,3 |
Germany-old |
2,0 |
2,0 |
1,9 |
1,4 |
1,3 |
1,2 |
Greece |
0,54 |
0,55 |
0,53 |
0,14 |
0,14 |
0,13 |
Hungary |
4,4 |
4,3 |
4,3 |
0,76 |
0,76 |
0,73 |
Ireland |
0,21 |
0,21 |
0,20 |
0,068 |
0,065 |
0,051 |
Italy |
1,5 |
1,5 |
1,5 |
0,71 |
0,71 |
0,63 |
Latvia |
0,41 |
0,40 |
0,37 |
0,26 |
0,26 |
0,23 |
Lithuania |
1,1 |
1,1 |
1,1 |
0,57 |
0,57 |
0,52 |
Luxembourg |
0,81 |
0,81 |
0,79 |
0,10 |
0,10 |
0,10 |
Macedonia |
0,51 |
0,51 |
0,50 |
0,19 |
0,19 |
0,19 |
Moldova |
0,69 |
0,69 |
0,68 |
0,54 |
0,54 |
0,52 |
Netherlands |
0,85 |
0,83 |
0,79 |
0,94 |
0,94 |
0,92 |
Norway |
0,26 |
0,25 |
0,21 |
0,10 |
0,10 |
0,078 |
Poland |
2,6 |
2,5 |
2,5 |
1,2 |
1,2 |
1,0 |
Portugal |
3,5 |
3,5 |
3,4 |
1,0 |
1,1 |
1,0 |
Romania |
2,1 |
2,1 |
2,1 |
0,29 |
0,29 |
0,28 |
Russia-Kaliningrad |
0,21 |
0,21 |
0,21 |
0 |
0 |
0 |
Russia-Kola/Karelia |
0,020 |
0,018 |
0,005 |
0 |
0 |
0 |
Remaining Russia |
0,91 |
0,90 |
0,88 |
0,17 |
0,17 |
0,14 |
Russia-St.Petersburg |
0,035 |
0,023 |
0,007 |
0 |
0 |
0 |
Slovakia |
3,4 |
3,4 |
3,4 |
0,72 |
0,72 |
0,71 |
Slovenia |
1,2 |
1,2 |
1,2 |
0,25 |
0,25 |
0,22 |
Spain |
2,3 |
2,3 |
2,2 |
0,63 |
0,64 |
0,56 |
Sweden |
1,0 |
1,0 |
0,89 |
0,36 |
0,37 |
0,31 |
Switzerland |
2,3 |
2,2 |
2,2 |
0,45 |
0,45 |
0,40 |
Ukraine |
2,0 |
2,0 |
2,0 |
0,68 |
0,67 |
0,59 |
United Kingdom |
1,0 |
1,0 |
1,0 |
1,3 |
1,3 |
1,2 |
Yugoslavia |
1,6 |
1,6 |
1,6 |
0,21 |
0,21 |
0,21 |
Atlantic Ocean |
0,52 |
0,52 |
0,51 |
0,036 |
0,036 |
0,036 |
Baltic Sea |
0,54 |
0,53 |
0,52 |
0,038 |
0,038 |
0,022 |
North Sea |
1,2 |
1,1 |
1,1 |
0,20 |
0,21 |
0,17 |
Table 6.2. Site-dependent characterisation factors for exposure of humans to photochemical ozone expressed as cumulative AOT60 (persppmhours/g). Factors calculated for the emission patterns of 1990
and 1995 and the expected pattern of 2010.
|
NOx |
VOC |
Country |
1990 |
1995 |
2010 |
1990 |
1995 |
2010 |
Albania |
9,0E-06 |
9,4E-06 |
2,0E-06 |
1,7E-06 |
4,0E-06 |
9,9E-07 |
Austria |
8,0E-05 |
7,0E-05 |
3,7E-05 |
8,6E-05 |
4,7E-05 |
5,0E-05 |
Belarus |
4,4E-06 |
2,5E-06 |
8,5E-07 |
7,4E-06 |
7,2E-09 |
3,0E-06 |
Belgium |
4,2E-04 |
3,8E-04 |
3,2E-04 |
2,9E-04 |
2,2E-04 |
2,0E-04 |
Bosnia/Herzeg. |
1,3E-05 |
1,3E-05 |
3,2E-06 |
3,8E-06 |
3,5E-05 |
2,6E-06 |
Bulgaria |
2,4E-06 |
2,2E-06 |
-2,4E-07 |
6,4E-06 |
2,2E-06 |
2,8E-06 |
Croatia |
3,8E-05 |
3,8E-05 |
1,6E-05 |
2,8E-05 |
1,2E-04 |
2,1E-05 |
Czech Republic |
2,3E-04 |
2,1E-04 |
1,7E-04 |
1,5E-04 |
6,2E-07 |
9,0E-05 |
Denmark |
4,0E-05 |
3,4E-05 |
2,2E-05 |
6,2E-05 |
2,7E-05 |
3,8E-05 |
Estonia |
1,4E-06 |
1,2E-06 |
6,4E-08 |
3,7E-07 |
9,4E-06 |
3,7E-07 |
Finland |
1,6E-06 |
8,5E-07 |
1,7E-07 |
1,8E-07 |
5,2E-07 |
1,0E-07 |
France |
2,4E-04 |
2,2E-04 |
1,6E-04 |
1,5E-04 |
1,2E-04 |
7,6E-05 |
Germany-new |
1,9E-04 |
1,7E-04 |
1,2E-04 |
2,1E-04 |
1,1E-04 |
1,1E-04 |
Germany-old |
3,6E-04 |
3,3E-04 |
2,7E-04 |
2,4E-04 |
1,9E-04 |
1,4E-04 |
Greece |
1,9E-05 |
1,9E-05 |
1,4E-05 |
1,6E-05 |
1,1E-05 |
1,3E-05 |
Hungary |
3,6E-05 |
3,0E-05 |
1,2E-05 |
5,2E-05 |
2,4E-05 |
3,1E-05 |
Ireland |
1,5E-05 |
1,2E-05 |
8,2E-06 |
1,5E-05 |
8,5E-06 |
9,7E-06 |
Italy |
2,0E-04 |
2,0E-04 |
1,4E-04 |
1,2E-04 |
1,0E-04 |
6,6E-05 |
Latvia |
3,8E-06 |
2,5E-06 |
1,7E-06 |
1,0E-06 |
1,0E-06 |
1,0E-06 |
Lithuania |
5,7E-06 |
4,2E-06 |
1,2E-06 |
3,4E-06 |
1,3E-06 |
1,4E-06 |
Luxembourg |
1,2E-04 |
1,1E-04 |
8,6E-05 |
6,6E-05 |
5,8E-05 |
4,9E-05 |
Macedonia |
4,1E-06 |
4,3E-06 |
0 |
0 |
3,8E-05 |
0 |
Moldova |
2,5E-06 |
1,5E-06 |
-4,9E-07 |
1,5E-06 |
1,8E-06 |
-8,0E-08 |
Netherlands |
2,5E-04 |
2,3E-04 |
1,8E-04 |
1,9E-04 |
1,3E-04 |
1,4E-04 |
Norway |
3,6E-06 |
2,1E-06 |
6,5E-07 |
1,9E-06 |
1,5E-06 |
9,5E-07 |
Poland |
1,2E-04 |
1,1E-04 |
8,6E-05 |
1,1E-04 |
6,9E-05 |
6,1E-05 |
Portugal |
1,3E-04 |
1,3E-04 |
9,7E-05 |
6,7E-05 |
6,7E-05 |
4,4E-05 |
Romania |
7,0E-06 |
5,0E-06 |
2,2E-08 |
1,3E-05 |
5,0E-06 |
5,7E-06 |
Russia-Kaliningrad |
4,4E-06 |
3,6E-06 |
2,4E-06 |
8,3E-07 |
4,7E-06 |
8,4E-07 |
Remaining Russia |
2,4E-06 |
2,1E-06 |
1,0E-06 |
1,8E-06 |
2,9E-06 |
5,3E-07 |
Russia-St.Petersburg |
5,9E-07 |
5,0E-07 |
2,0E-07 |
-1,1E-07 |
2,9E-05 |
0 |
Slovakia |
6,3E-05 |
5,6E-05 |
4,0E-05 |
5,5E-05 |
1,5E-06 |
3,2E-05 |
Slovenia |
2,6E-05 |
2,6E-05 |
1,4E-05 |
1,6E-05 |
2,7E-06 |
1,3E-05 |
Spain |
4,8E-05 |
4,6E-05 |
2,4E-05 |
2,7E-05 |
2,4E-05 |
1,4E-05 |
Sweden |
1,5E-05 |
1,2E-05 |
6,0E-06 |
1,4E-05 |
8,3E-06 |
9,2E-06 |
Switzerland |
1,1E-04 |
9,8E-05 |
4,8E-05 |
1,0E-04 |
6,1E-05 |
5,2E-05 |
Ukraine |
5,6E-06 |
3,7E-06 |
5,0E-07 |
2,3E-05 |
2,1E-07 |
8,9E-06 |
United Kingdom |
1,1E-04 |
9,9E-05 |
8,5E-05 |
9,5E-05 |
6,0E-05 |
9,4E-05 |
Yugoslavia |
3,4E-06 |
2,2E-06 |
-1,5E-06 |
2,9E-06 |
1,4E-05 |
1,7E-06 |
Atlantic Ocean |
1,4E-05 |
1,4E-05 |
8,6E-06 |
0 |
0 |
0 |
Baltic Sea |
1,9E-06 |
1,5E-06 |
7,4E-07 |
0 |
0 |
0 |
North Sea |
8,7E-05 |
7,8E-05 |
5,8E-05 |
0 |
0 |
0 |
Site-generic characterisation factors
From the site-dependent characterisation factors of Table 6.1 and 6.2 site-generic factors are calculated as the emission-weighted average over all the countries and regions. The site-generic factors are
compatible with the site-dependent factors in the sense that they are calculated using the same model and therefore cover the same part of the causality chain underlying photochemical ozone formation
impacts. The only difference is that the spatial variation in the included parameters is no longer represented. The site-generic factors can be used if spatial differentiation is unwanted or if the location of some
of the processes in the product system is unknown or lies outside Europe. The standard deviation of the site-generic factors reflects the spatially determined variation underlying the site-generic factors.
Table 6.3. Site-generic characterisation factors for exposure of vegetation to photochemical ozone expressed as cumulative AOT40 in exceedance of the critical level (m2ppmhours/g). The factors are
calculated as the emission-weighted means of site-dependent factors in Table 6.1 for the emission patterns of 1990 and 1995 and the expected pattern of 2010.
|
NOx |
VOC |
Year |
1990 |
1995 |
2010 |
1990 |
1995 |
2010 |
Average |
1,76 |
1,76 |
1,63 |
0,74 |
0,73 |
0,61 |
Standard deviation |
2,83 |
2,87 |
2,26 |
1,31 |
1,21 |
1,02 |
Table 6.4. Site-generic characterisation factors for exposure of humans to photochemical ozone expressed as cumulative AOT60 (persppmhours/g). The factors are calculated as the emission-weighted
means of site-dependent factors in Table 6.2 for the emission patterns of 1990 and 1995 and the expected pattern of 2010.
|
NOx |
VOC |
Year |
1990 |
1995 |
2010 |
1990 |
1995 |
2010 |
Average |
1,3E-04 |
1,2E-04 |
1,1E-04 |
8,7E-05 |
5,9E-05 |
7,6E-05 |
Standard deviation |
2,9E-04 |
2,7E-04 |
2,3E-04 |
1,7E-04 |
1,3E-04 |
1,4E-04 |
6.4.1 Sub categories of ozone formation
Measures of the AOT40 and AOT60 are by no means proportional, and a closer study of the factors for vegetation exposure and for human exposure in Tables 6.1 and 6.2 reveals marked differences in the
ranking and relative impact of different emission countries. It is therefore concluded that the exposure of humans and vegetation must be described by each their index and the impact category is split into two
sub categories representing respectively:
- critical ozone exposure of vegetation
- critical ozone exposure of humans
Being defined earlier in the cause-impact chain, the EDIP97 impact category photochemical ozone formation covers all impacts from photo-oxidants. In addition to human health and vegetation impacts, also
damage to man-made materials is thus represented by the POCP-based impact potential. None of the two sub categories introduced here explicitly covers material damage. Indeed, it is mentioned in Heyes
et al., 1997b, that the UN/ECE has proposed a preliminary threshold of an annual mean ozone concentration of 20 ppb based on an acceptable deterioration rate for sensitive organic materials. Separate
calculations for material damage require additional information of the distribution of sensitive materials over the grids of the RAINS model. This far, no model calculations have been performed for material
damage but until that may happen we suggest to consider damage to materials represented under the human health exposure. Even though the suggested threshold for materials is different, the geographic
distribution of sensitive man-made materials is likely to be well represented by the geographic population distribution as already included in the RAINS model.
6.4.2 Characteristics of the new characterisation factors
The site-dependent characterisation factors presented in Tables 6.1 and 6.2 and the site-generic factors in Tables 6.3 and 6.4 are calculated using a model that covers a larger part of the cause-impact chain
in Figure 6.1. In contrast to the old factors that nearly exclusively represent substance-specific characteristics, the new factors also include the dispersion, the concentration increase and the background
concentration in the receptor area, the population density or density of vegetation in the receptor area as well as some quantitative concentration-effect relationship for vegetation. The new factors thus
eliminate a large part of the spatially determined uncertainty that is included in the EDIP97 factors.
Reflecting the actual emission patterns of European countries, the new factors are dependent on changes in these patterns. Therefore, the factors have been calculated for the emission patterns of 1990, 1995
and the expected pattern of 2010. For vegetation exposure, the temporal variability is negligible for most emitter countries but for human exposure, there are significant differences depending on the basis
year. In the guideline, the factors based on 1995 emissions is suggested as the default but for emissions taking place in the future, e.g. from the late use stage or disposal stage of the product, the factors
based on 2010 could be applied alternatively as part of the sensitivity analysis.
The new factors also include the contribution from NOx. From Tables 6.3 and 6.4 it is clear that on a weight basis, the factor for NOx is on average considerably larger than the factor for VOC, so this is
also an important improvement compared to the EDIP97 factors.
On the other hand, the new characterisation factors do not allow the differentiation between individual VOCs. This aspect is discussed in the next section.
6.4.3 Differentiation between individual VOCs
As mentioned in Section 6.3.1 the ozone formation model applied in RAINS does not allow distinction between individual VOCs. This eliminates a source of variation in the factors but since the variation
between the ozone formation potentials lies within a factor 2-4 for most VOCs, this potential error is small compared to the variation eliminated by including the contribution from NOx. Nonetheless, it is
suggested to introduce differentiation between the individual VOCs through an efficiency factor s representing the ozone formation potential of a specific VOC (s) relative to the average ozone formation
potential of European VOC emissions
(6.3)
s126
The European average POCP was determined for 1985 emissions at 0,40 in Hauschild and Wenzel (1998). Based on this figure and the POCP values applied for characterisation factors in EDIP97, the
efficiency factor is calculated and tabulated in Annex 6.3 for the most common individual VOCs as well as for source-specified VOC-mixtures frequently encountered in life cycle inventories.
The RAINS model only considers non-methane VOCs and NOx. In comparison, the POCP values used in EDIP97 and other life cycle impact assessment methodologies are also available for methane and
carbon monoxide. To include these two substances which may be of importance for some processes, the following assumptions are made:
Including carbon monoxide
In addition to being a substance emitted from processes with incomplete combustion, carbon monoxide is also an intermediary product in the photochemical oxidation of some VOCs and its atmospheric
lifetime is thus within the interval relevant for non-methane VOCs. It is therefore assumed that the dispersion and deposition pattern of carbon monoxide is well represented by the model applied for VOCs
and in the calculation of photochemical ozone impact, carbon monoxide is treated as a VOC.
Including methane
In contrast, methane is an extremely long-lived organic compound with an estimated residence time in the atmosphere of around 10 years. This means that a globally uniform distribution of methane in the
atmosphere must be expected and the contribution of methane to ozone formation is rather low at a regional level. In the calculation of characterisation factors it is suggested to base the characterisation
factors for methane on the site-generic factors developed for VOCs and correct for the fact that due to the long lifetime of methane, a large part of the ozone formed will expose ocean areas and hence not
contribute to exposure of vegetation or humans. A correction factor of 0,5 is proposed.
Table 6.5. Site-generic characterisation factors for methane proposed to represent the exposure of vegetation or humans to photochemical ozone. The factors are based on the VOC factors in Table 6.3 and
6.4
|
Vegetation exposure
(m2ppmhours)
|
Human exposure
(persppmhours)
|
Year |
1990 |
1995 |
2010 |
1990 |
1995 |
2010 |
Methane |
0,37 |
0,36 |
0,31 |
4,410-5 |
2,910-5 |
3,810-5 |
6.5 How to perform site-generic and site-dependent characterisation
The site-generic photochemical ozone impacts from a product are calculated applying the new site-generic characterisation factors of Table 6.3, 6.4 or 6.5 in the following formulas:
Click here to see the Figure.
Where:
sg POI(veg) is the site-generic photochemical ozone formation impact on vegetation expressed as area exposed above threshold (in m2ppmhours/f.u.)
sg POI(hum) is the site-generic photochemical ozone formation impact on human health expressed as persons exposed above threshold (in persppmhours/f.u.)
sg POF(veg)VOC is the site-generic photochemical ozone formation factor from Table 6.3 that relates emission of VOCs or CO to the impact on vegetation in the deposition area (in m2ppmhours/g).
sg POF(veg)NOx is the site-generic photochemical ozone formation factor for from Table 6.3 that relates emission of NOx to the impacts on vegetation in the deposition area (in m2ppmhours/g).
sg POF(hum)VOC is the site-generic photochemical ozone formation factor from Table 6.4 that relates emission of VOCs or CO to the impacts on human health in the deposition area (in persppmhours/g).
sg POF(hum)NOx is the site-generic photochemical ozone formation factor from Table 6.4 that relates emission of NOx to the impacts on human health in the deposition area (in persppmhours/g).
sg POF(veg)CH4 is the site-generic photochemical ozone formation factor for from Table 6.5 that relates emission of CH4 to the impacts on vegetation in the deposition area (in m2ppmhours/g).
sg POF(hum)CH4 is the site-generic photochemical ozone formation factor from Table 6.5 that relates emission of CH4 to the impacts on human health in the deposition area (in persppmhours/g).
is a substance-specific efficiency factor from Annex 6.3 expressing the ozone creation potential of the individual volatile organic compound or CO (s) or methane relative to the ozone creation potential of the
European average VOC (dimensionless).
E is the emission of NOx, CH4 or individual or source-specified VOC or CO (s) according to index (in g/f.u.)
The potential spatially determined variation of the site-generic photochemical ozone impacts, can be estimated from the standard deviation given in Table 6.3, 6.4 or 6.5 for each substance.
The site-dependent photochemical ozone impacts from a process in the life cycle of a product are calculated applying the new site-dependent characterisation factors of Table 6.1 and 6.2 in the following
formulas:
Click here to see the Figure.
Where:
sd POI(veg)p is the site-dependent photochemical ozone formation impact on vegetation expressed as area exposed above threshold by the selected process (p) (in m2ppmhours/f.u.)
sd POI(hum)p is the site-dependent photochemical ozone formation impact on human health expressed as persons exposed above threshold by the selected process (p) (in persppmhours/f.u.)
sd POF(veg)NOx,i is the site-dependent photochemical ozone formation factor from Table 6.1 that relates emission of NOx from country or region (i), where the selected process (p) is located, to the
impacts on vegetation in the deposition area (in m2ppmhours/g).
sd POF(veg)VOC,i is the site-dependent photochemical ozone formation factor from Table 6.1 that relates emission of VOCs or CO from country or region (i), where the selected process (p) is located, to
the impact on vegetation in the deposition area (in m2ppmhours/g).
sg POF(veg)CH4 is the site-generic photochemical ozone formation factor CH4 from Table 6.4 that relates emission of CH4 to the impacts on vegetation in the deposition area (in m2ppmhours/g).
sd POF(hum)NOx,i is the site-dependent photochemical ozone formation factor from Table 6.2 that relates emission of NOx from country or region (i), where the selected process (p) is located, to the
impacts on human health in the deposition area (in persppmhours/g).
sd POF(hum)VOC,p is the site-dependent photochemical ozone formation factor from Table 6.2 that relates emission of VOCs or CO from country or region (i), where the selected process (p) is located,
to the impacts on human health in the deposition area (in persppmhours/g).
sg POF(hum)CH4 is the site-generic photochemical ozone formation factor from Table 6.5 that relates emission of CH4 to the impacts on human health in the deposition area (in persppmhours/g).
η is a substance-specific efficiency factor from Annex 6.3 expressing the ozone creation potential of the individual volatile organic compound or CO (s) or methane relative to the ozone creation potential of the
European average VOC (dimensionless).
Ep is the emission of NOx, CH4 or individual or source-specified VOC or CO (s), according to index, from process (p) (in g/f.u.)
Emissions from a non-European or unknown region may as a first approach be calculated using the site-generic factors from Table 6.3, 6.4 or 6.7. The standard deviations on the site-generic factors in these
tables give a range of potential spatial variation for the application of the site-generic factor within Europe. Given the size of the variation in emissions and sensitivities within Europe, the site-dependent factor
is expected to lie within this range for most regions also in the rest of the world. Expert judgement may be used in the interpretation to assess whether the factor for emissions from processes in non-European
regions should be found in the lower or upper end of the range.
6.6 Normalisation references
The normalisation reference for photochemical ozone formation is calculated using the new characterisation factors on the national European emission inventories for the corresponding year. Three sets of
references are calculated in Annex 6.4 and the reference based on 1995 factors and emissions is retained as the EDIP default reference for normalisation in life cycle impact assessment.
Table 6.6. Normalisation references for the two sub categories of photochemical ozone formation calculated for the reference yeras 1990, 1995 and 2010 in Annex 6.4 and expressed as impact per person
per year (the person equivalent).
| Vegetation exposure (m2ppmhours/pers/yr)
| Human exposure (persppmhours/pers/yr)
|
Year | 1990 | 1995 | 2010 | 1990 | 1995 | 2010 |
Person equivalent | 1,6105 | 1,4105 | 0,87105 | 13 | 10 | 4,6 |
From Table 6.6 it is seen that the photochemical ozone impact was reduced from 1990 to 1995 and is expected to continue the trend towards 2010.
6.7 Interpretation and recommendations
The new photochemical ozone formation impact potentials are improved in two aspects compared to the impact potentials calculated using the EDIP97 characterisation factors or other systems based on the
POCP or MIR approach; the environmental relevance is increased and a part of the spatial variation in sensitivity of the receiving environment is now taken into account.
Environmental relevance
The environmental relevance is increased because the exposure of the sensitive parts of the environment (vegetation or human beings) is included in the underlying model which now covers most of the
causality chain towards the LCA protection areas: Ecosystem health and human health. This is particularly important when weighting factors based on the environmental relevance are used instead of the
EDIP default weighting factors (which are based on political reduction targets that for photochemical ozone formation are also aiming for protection of vegetation and human health). In comparison, the
EDIP97 factors only cover the potential for formation of ozone.
In addition, the contribution of NOx is now included in the impact potentials. The significance of this for a specific product system depends on the quantities of NOx and VOCs emitted. From the calculation
of the normalisation references, it is known that on a European level, NOx contributes around twice as much as VOC to photochemical ozone formation and on average the characterisation factor for NOx is
more than three times the characterisation factor of VOCs.
Spatial variation
The spatial variation in exposure for photochemical ozone formation can be large even at the very local scale. The variation in sensitivity between European regions are now presented on a national scale
showing a factor 15-20 of difference between least and the most sensitive emission countries for exposure of vegetation and a factor of around 400 times of difference for exposure of humans (the latter
reflecting the variation in population density in the deposition areas). This variation is hidden when the EDIP97 factors or similar are used for characterisation.
6.8 References
Alcamo, J., R. Shaw and L. Hordijk (eds.). The RAINS model of acidification. Science and strategies in Europe. Dordrecht (the Netherlands), Kluwer Academic Publishers, 1990.
Amann, M., M. Baldi, C. Heyes, Z. Klimont and W. Schöpp. Integrated assessment of emission control scenarios, including the impact of troposheric ozone. Water, air and soil pollution, 85(4),
2595-2600, 1995.
Andersson-Sköld, Y., Grennfelt, P., and Pleijel, K.: Photochemical ozone creation potentials: a study of different concepts. J. Air Waste Manage. Assoc. 42(9), 1152-1158, 1992.
Derwent, R.G., and Jenkin, M.E.: Hydrocarbon involvement in photochemical ozone formation in Europe. AERE R 13736, AEA Environment and Energy, Harwell Laboratory, Oxfordshire, UK, 1990.
Derwent, R.G., Grennfelt, G., and Hov, Ø.: Photochemical oxidants in the atmosphere. Nordic Council of Ministers, Nord 1991:7, Copenhagen, 1991.
Derwent, R.G., Jenkin, M.E., Saunders, S.M., et al.: Photochemical ozone creation potentials for organic compounds in northwest Europe calculated with a master chemical mechanism. ATMOS
ENVIRON 32 (14-15): 2429-2441, 1998
Granby, K., Kemp, K., Palmgren, F., Hovmand, M. and Egeløv, A.: Measurements of Photochemical Pollutants. In: Fenger, J. (ed.): Photochemical Air Pollution. Danish Aspects. National Environmental
Research Institute. - NERI Technical Report 199: 63-101, 1997.
Hauschild, M. and Wenzel, H.: Photochemical ozone formation as criterion the environmental assessment of products. In Hauschild, M.Z. and Wenzel, H.: Environmental assessment of products. Vol. 2 -
Scientific background, 565 pp. Chapman & Hall, United Kingdom, ISBN 0412 80810 2, Kluwer Academic Publishers, Hingham, MA. USA., 1998.
Hettelingh J.-P., Posch M., de Smet P., Downing R.J.: The use of critical loads for
emission reduction agreements in Europe. Water, Air and Soil Pollution 85:2381-2388, 1995.
Heyes, C., Schöpp, W., Amann: A simplified model to predict long-term ozone concentrations in Europe. WP-96-12, International Institute for Applied Systems Analysis (IIASA), Laxenburg, Austria,
1996.
Heyes, C., Schöpp, W., Amann, M., Bertok, I., Cofala, J., Gyarfas, F., Klimont, Z., Makowski, M. and Shibayev, S.: A model for optimizing strategies for controlling ground-level ozone in Europe. Interim
report IR-97-002/January, International Institute for Applied Systems Analysis (IIASA), Laxenburg, Austria, 1997a.
Heyes, C., Schöpp, W., Amann, M., Bertok, I., Cofala, J., Gyarfas, F., Klimont, Z., Makowski, M. and Shibayev, S.: Simultaneous optimization of abatement strategies for ground-level ozone and
acidification. Interim report IR-97-090/December, International Institute for Applied Systems Analysis (IIASA), Laxenburg, Austria, 1997b.
Kärenlampi L. and Skarby L., (eds), 1996. Critical levels for Ozone in Europe: Testing and
Finalizing the Concepts. UN-ECE Workshop Report. Dept. of Ecology and Environmental Science, University of Kuopio, Finland, 1996.
Larsen, P.B., Larsen, J.C., Fenger, J., and Jensen, S.S.: Health evaluation of air pollution from road traffic (In Danish: Sundhedsmæssig vurdering af luftforurening fra vejtrafik). Miljøprojekt nr. 352, Danish
Environmental Protection Agency, Copenhagen, 1997.
Lübkert, B. and Schöpp, W.: A model to calculate natural (volatile organic compounds) VOC emissions from forests in Europe, wp-89-82, 65 pp, w89082, IIASA, Laxenburg, Austria, 1989
Posch, M., Hettelingh, J.P., Downing, R.J. and P.A.M. de Smet (eds): Calculation and mapping of critical thresholds in Europe. CCE status report 1997. Co-ordination Centre for Effects (CCE) at the
National Institute of Public Health and the Environment (RIVM), Bilthoven (the Netherlands), 1997.
Wenzel, H., Hauschild M.Z. and Alting, L.: Environmental assessment of products. Vol. 1 - Methodology, tools, techniques and case studies, 544 pp. Chapman & Hall, United Kingdom, ISBN 0 412
80800 5, Kluwer Academic Publishers, Hingham, MA. USA., 1997.
World Health Organization: Indoor air quality: organic pollutants. EURO Reports and Studies 111, WHO Regional Office for Europe, Copenhagen, 1989
WHO: Ozone. In: Air Quality Guidelines for Europe, pp 315-326. WHO Regional Publications, European Series No 23, Copenhagen, 1987
Annex 6.1 Influence from meteorological conditions
Calculation of AOT60 for individual European countries based on 1995 emissions and meteorological data for five different years.
Click here to see the Table.
Annex 6.2 POCP values for individual VOCs
POCP values from different sources (Derwent and Jenkin, 1990, Derwent et al., 1998, Andersson-Skjöld et al., 1992). EDIP97 applies the low NOx values from Andersson-Skjöld et al., 1992 for
emissions from low NOx areas and the values from Derwent and Jenkin, 1990 for high NOx areas. In EDIP97, all values are scaled down by a factor 100 so the reference substance ethylene has a POCP
of 1,00.
|
Hydrocarbon |
POCP
(Derwent and Jenkin, 1990)
|
POCP
Derwent et al.,
1998
|
POCP,
low NOx, 4 days
(Andersson-Skjöld et al., 1992)
|
POCP
high NOx, 4 days (Andersson-Skjöld et al., 1992)
|
ALKANES |
methane |
0,7 |
0,6 |
|
|
|
ethane |
8,2 |
12,3 |
12,6 |
12,1 |
|
propane |
42,1 |
17,6 |
50,3 |
51,8 |
|
n-butane |
41,4 |
35,2 |
46,7 |
48,5 |
|
i-butane |
31,5 |
30,7 |
41,1 |
38,9 |
|
n-pentane |
40,8 |
39,5 |
29,8 |
38,7 |
|
i-pentane |
29,6 |
40,5 |
31,4 |
34,5 |
|
Neopentane |
|
17,3 |
|
|
|
n-hexane |
42,1 |
48,2 |
45,2 |
49,5 |
|
2-methylpentane |
52,4 |
42 |
52,9 |
56,5 |
|
3-methylpentane |
43,1 |
47,9 |
40,9 |
45,7 |
|
2,2-dimethylbutane |
25,1 |
24,1 |
|
|
|
3,2-dimethylbutane |
38,4 |
54,1 |
|
|
|
n-heptane |
52,9 |
49,4 |
51,8 |
59,2 |
|
2-methylhexane |
49,2 |
41,1 |
|
|
|
3-methylhexane |
49,2 |
36,4 |
|
|
|
n-octane |
49,3 |
45,3 |
46,1 |
54,4 |
|
2-methylheptane |
46,9 |
|
45,7 |
52,4 |
|
n-nonane |
46,9 |
41,4 |
35,1 |
46,3 |
|
2-methyloctane |
50,5 |
|
45,4 |
52,3 |
|
n-decane |
46,4 |
38,4 |
42,2 |
50,9 |
|
2-methylnonane |
44,8 |
|
42,3 |
49,8 |
|
n-undecane |
43,6 |
38,4 |
38,6 |
47,6 |
|
n-dodecane |
41,2 |
35,7 |
31,1 |
45,2 |
|
methylcyclohexane |
58 |
|
50,2 |
58 |
CYCLOHEXANES |
cyclohexane |
|
29 |
|
|
|
cyclohexanone |
|
29,9 |
|
|
|
cyclohexanol |
|
44,6 |
|
|
ALKENES |
ethylene |
100 |
100 |
100 |
100 |
|
propylene |
103 |
112,3 |
59,9 |
106 |
|
1-butene |
95,9 |
107,9 |
49,5 |
98,3 |
|
cis-but-2-ene |
|
114,6 |
|
|
|
2-butene (trans) |
99,2 |
113,2 |
43,6 |
102,1 |
|
methylpropene |
|
62,7 |
|
|
|
1-pentene |
105,9 |
97,7 |
42,4 |
83,3 |
|
cis-pent-2-ene |
|
112,1 |
|
|
|
2-pentene (trans) |
93 |
111,7 |
38,1 |
96,5 |
|
2-methylbut-1-ene |
77,7 |
77,1 |
18,1 |
71,7 |
|
2-methylbut-2-ene |
77,9 |
84,2 |
|
|
|
3-methylbut-1-ene |
89,5 |
67,1 |
45,3 |
78,4 |
|
hex-1-ene |
|
87,4 |
|
|
|
cis-hex-2-ene |
|
106,9 |
|
|
|
trans-hex-2-ene |
|
107,3 |
|
|
|
styrene |
|
14,2 |
|
|
DIALKENES |
1,3-butadiene |
|
85,1 |
|
|
|
butylen=isobutene
=2-methylpropene
|
64,3 |
|
58 |
64,8 |
|
isoprene (C5H8) |
76,8 |
109,2 |
58,3 |
76,8 |
ALKYNES |
acetylene |
16,8 |
8,5 |
36,8 |
29,1 |
AROMATICS |
benzene |
18,9 |
21,8 |
40,2 |
31,8 |
|
toluene |
56,3 |
63,7 |
47 |
56,5 |
|
o-xylene |
66,6 |
105,3 |
16,7 |
59,8 |
|
m-xylene |
99,3 |
110,8 |
47,4 |
88,4 |
|
p-xylene |
88,8 |
101 |
47,2 |
79,6 |
|
ethylbenzene |
59,3 |
73 |
50,4 |
62,1 |
|
propylbenzene |
|
63,6 |
|
|
|
1,2,3-trimethylbenzene |
117 |
126,7 |
29,2 |
86,8 |
|
1,2,4-trimethylbenzene |
120,3 |
127,8 |
33 |
93,8 |
|
1,3,5-trimethylbenzene |
114,5 |
138,1 |
33 |
98,9 |
|
o-ethyltoluene |
66,8 |
89,8 |
40,8 |
63,7 |
|
m-ethyltoluene |
79,4 |
101,9 |
40,1 |
72,9 |
|
p-ethyltoluene |
72,5 |
90,6 |
44,3 |
68,1 |
|
n-propylbenzene |
|
|
45,4 |
53,1 |
|
iso-propylbenzene |
|
50 |
52,3 |
59,4 |
|
3,5-dimethylethylbenzene |
|
132 |
|
|
|
3,5-diethyltoluene |
|
129,5 |
|
|
ALDEHYDES |
formaldehyde |
42,1 |
51,9 |
26,1 |
37,9 |
|
acetaldehyde |
52,7 |
64,1 |
18,6 |
61,5 |
|
propionaldehyd |
60,3 |
79,8 |
17 |
65,2 |
|
butyraldehyd |
56,8 |
79,5 |
17,1 |
59,7 |
|
i-butyraldehyd |
63,1 |
51,4 |
30 |
67,7 |
|
pentanaldehyd |
|
76.5 |
|
|
|
valeraldehyd |
68,6 |
|
32,1 |
68,6 |
|
acroleine (CH2CHCHO) |
82,7 |
|
82,3 |
82,7 |
|
benzaldehyd |
-33,4 |
-9,2 |
|
|
KETONES |
acetone |
17,8 |
9,4 |
12,4 |
16 |
|
methylethylketone |
42,3 |
37,3 |
17,8 |
34,6 |
|
methyl i-butyl ketone |
63,3 |
49 |
31,8 |
66,6 |
|
methylpropylketone |
|
54,8 |
|
|
|
diethylketone |
|
41,4 |
|
|
|
methylipropylketone |
|
36,4 |
|
|
|
hexan2one |
|
57,2 |
|
|
|
hexan3one |
|
59,9 |
|
|
|
methyltbutylketone |
|
32,3 |
|
|
ALCOHOLS |
methanol |
12,3 |
13,1 |
21,3 |
17,8 |
|
ethanol |
26,8 |
38,6 |
22,5 |
31,7 |
|
2-methoxyethanol |
|
30 |
|
|
|
2-ethoxyethanol |
|
38,7 |
|
|
|
1-butoxypropanol |
|
43,6 |
|
|
|
2-butoxyethanol |
|
43,8 |
|
|
|
1-methoxy-2-propanol |
|
36,8 |
|
|
|
i-propanol |
18,8 |
14 |
20,3 |
18,8 |
|
n-propanol |
|
54,3 |
|
|
|
n-butanol |
|
61,2 |
|
|
|
butanol |
40,4 |
|
21,4 |
40,4 |
|
i-butanol |
29 |
37,5 |
25,5 |
29 |
|
s-butanol |
|
40 |
|
|
|
t-butanol |
|
12,3 |
|
|
|
3-pentanol |
|
42,2 |
|
|
|
2-methylbutan-1-ol |
|
40,7 |
|
|
|
3-methylbutan-1-ol |
|
41,2 |
|
|
|
3-methylbutan-2-ol |
|
36,6 |
|
|
|
2-methylbutan-2-ol |
|
14,2 |
|
|
|
diacetone alcohol |
|
26,2 |
|
|
|
butane-2-diol |
21,6 |
|
6,6 |
21,6 |
GLYCOLS |
propylene glycol |
|
45,7 |
|
|
|
ethylene glycol |
|
38,2 |
|
|
CARBOXYLIC ACIDS |
formic acid |
|
3,2 |
|
|
|
acetic acid |
|
9,7 |
|
|
|
propanoic acid |
|
15 |
|
|
ETHERS |
dimethyl ether |
28,6 |
17,4 |
34,6 |
28,6 |
|
methyl-t-butyl ether |
|
15,2 |
|
|
|
ethyl-t-butyl ether |
|
21,4 |
|
|
|
diethylether |
|
46,7 |
|
|
|
diisopropylether |
|
47,6 |
|
|
|
propylene glycol
methyl ether
|
49,7 |
45,7 |
49,1 |
49,7 |
ESTERS |
methyl formate |
|
3,3 |
|
|
|
methyl acetate
(=dimethyl ester)
|
2,5 |
4,6 |
6,7 |
4,6 |
|
ethyl acetate |
21,8 |
21,3 |
29,4 |
28,6 |
|
i-propyl acetate |
21,5 |
21,3 |
|
|
|
n-propyl acetate |
|
29,0 |
|
|
|
n-butyl acetate |
32,3 |
24,1 |
32 |
36,7 |
|
s-butyl acetate |
|
26,7 |
|
|
|
t-butyl acetate |
|
6,5 |
|
|
|
i-butyl acetate |
33,2 |
21,3 |
35,3 |
34,5 |
|
propylene glycol
methyl ether acetate
|
14,3 |
45,7 |
15,7 |
14,3 |
HALOCARBONS |
methyl chloride |
|
0.5 |
|
|
|
methylene chloride |
0,9 |
6,8 |
2,3 |
1,7 |
|
chloroform (CHCl3) |
0,3 |
2,3 |
0,4 |
0,3 |
|
methyl chloroform |
0,1 |
0,9 |
0,2 |
0,1 |
|
cis-dichloroethylene |
|
44,7 |
|
|
|
trans-dichloroethylene |
|
39,2 |
|
|
|
trichloroethylene |
6,6 |
32,5 |
11,1 |
9,1 |
|
tetrachloroethylene |
0,5 |
2,9 |
1,4 |
1 |
|
allyl chloride
(CH2CHCH2Cl)
|
67,7 |
|
48,3 |
67,7 |
CO |
carbon monoxide |
3,2 |
|
4 |
3,2 |
|
|
|
|
|
|
Annex 6.3 Efficiency factors for individual VOCs and source-specified VOCs
The dimensionless efficiency factor is representing the efficiency of individual VOCs relative to the European average VOC in contributing to ozone formation. It is derived as the quotient between the
respective POCP-factors for 4-9 days in high NOx-areas (the EDIP97 characterisation factors for high NOx-areas, Wenzel et al., 1997).
Individual VOCs and methane |
Efficiency
factor i
|
Individual VOCs, carbon monoxide and source-specified VOCs |
Efficiency
factor i
|
|
|
|
|
Alkanes |
1,0 |
Aldehydes |
1,5 |
methane |
0,018 |
formaldehyde |
1,1 |
ethane |
0,21 |
acetaldehyde |
1,3 |
propane |
1,1 |
propionaldehyde |
1,5 |
n-butane |
1,0 |
butyraldehyde |
1,4 |
isobutane |
0,79 |
isobutyraldehyde |
1,6 |
n-pentane |
1,0 |
valeraldehyde |
1,7 |
isopentane |
0,74 |
acrolein |
2,0 |
n-hexane |
1,1 |
Ketones |
1,0 |
2-methylpentane |
1,3 |
acetone |
0,45 |
3-methylpentane |
1,1 |
methyl ethyl ketone |
1,1 |
2,2-dimethylbutane |
0,63 |
methyl isobutyl ketone |
1,6 |
2,3-dimethylbutane |
0,96 |
Alcohols |
0,66 |
n-heptane |
1,3 |
methanol |
0,31 |
2-methylhexane |
1,2 |
ethanol |
0,67 |
3-methylhexane |
1,2 |
isopropanol |
0,50 |
n-octane |
1,2 |
butanol |
1,0 |
2-methylheptane |
1,2 |
isobutanol |
0,75 |
n-nonane |
1,2 |
butan-2-diol |
0,75 |
2-methyloctane |
1,3 |
Ethers |
1,0 |
n-decane |
1,2 |
dimethyl ether |
0,75 |
2-methylnonane |
1,1 |
propylene glycol methyl ether |
1,3 |
n-undecane |
1,1 |
Esters |
0,51 |
n-dodecane |
1,0 |
methyl acetate (=dimethyl ester) |
0,06 |
Alkenes |
2,2 |
ethyl acetate |
0,55 |
ethylene |
2,5 |
isopropyl acetate |
0,54 |
propylene |
2,6 |
n-butyl acetate |
0,81 |
1-butene |
2,4 |
isobutyl acetate |
0,83 |
2-butene (trans) |
2,5 |
Propylene glycol methyl ether acetate |
0,25 |
Isobutene |
1,5 |
Chloro-alkanes |
0,011 |
2-pentene (trans) |
2,3 |
methylene chloride |
0,023 |
1-pentene |
2,6 |
chloroform |
0,0075 |
2-methylbut-1-ene |
1,9 |
methyl chloroform |
0,0025 |
3-methylbut-1-ene |
2,2 |
Chloro-alkenes |
0,64 |
2-methylbut-2-ene |
1,9 |
trichloroethylene |
0,17 |
2-methylpropene |
1,6 |
tetrachloroethylene |
0,01 |
Isoprene |
2,0 |
allyl chloride |
1,8 |
Alkynes |
|
Inorganic compounds |
|
acetylene |
0,42 |
carbon monoxide |
0,075 |
Aromatics |
1,9 |
|
|
benzene |
0,47 |
Source-specified VOC mixtures |
|
toluene |
1,4 |
Petrol-powered car, exhaust |
1,5 |
o-xylene |
1,7 |
Petrol-powered car, vapour |
1,3 |
m-xylene |
2,5 |
Diesel-powered car, exhaust |
1,5 |
p-xylene |
2,2 |
Power plants |
1,3 |
ethylbenzene |
1,5 |
Combustion of wood |
1,5 |
n-propylbenzene |
1,2 |
Food industry |
1,0 |
isopropylbenzene |
1,4 |
Surface coating |
1,3 |
1,2,3-trimethylbenzene |
2,9 |
Chemical cleaning of clothes |
0,75 |
1,2,4-trimethylbenzene |
3,0 |
Refining and distribution of oil |
1,3 |
1,3,5-trimethylbenzene |
2,9 |
Natural gas leakage |
0,050 |
o-ethyltoluene |
1,7 |
Coal mining |
0,018 |
m-ethyltoluene |
2,0 |
Framing |
1,0 |
p-ethyltoluene |
1,8 |
Landfilling of household waste |
0,018 |
Annex 6.4 Normalisation references for photochemical ozone formation
Normalisation references for photochemical ozone formation calculated with the new characterisation factors and the national European emission inventories for each of the years 1990, 1995 and 2010.The
reference based on 1995 factors and emissions is retained as default factor for normalisation in life cycle impact assessment.
Impacts on vegetation
Click here to see the Table.
Footnotes
[44] Institute of Product Development (IPU) in Denmark
[45] National Environmental Research Institute (NERI) in Denmark
[46] International institute for Applied Systems Analysis (IIASA) in Austria
| Front page | | Contents | | Previous | | Next | | Top |
Version 1.0 April 2005, © Danish Environmental Protection Agency
|